When it comes to endurance activities—be it mountaineering, trail running, or ski touring—a thorough understanding of how the body produces and uses energy can give athletes a distinct performance advantage. I’ve always personally been motivated by understanding the why behind what I’m doing in my training. Central to our understanding of how training works are the mitochondria, which not only generate energy through aerobic pathways but also tap into lactate as a fuel source.
Although lactate was once cast in a negative light—often blamed for the “burn” that accompanies intense exercise—we know now that it plays a crucial role as a readily oxidizable substrate (read: fuel). By training both the aerobic system and the pathways responsible for lactate metabolism, athletes can strategically increase endurance, delay fatigue, and recover better, leading to better performance in mountain and trail environments.
ALL ABOUT MITOCHONDRIA
In this post I want to delve into the microscopic world of your mitochondria. One of the primary ways endurance training enhances performance is by driving mitochondrial adaptations at the cellular level.
Mitochondria, often called the “powerhouses” of the cell, are responsible for aerobic energy production. Over the course of a systematic endurance training program, such as one built for you by a coach or one of our proven training plans, these organelles undergo a process known as mitochondrial biogenesis, meaning they which leads to a greater number of mitochondria per muscle fiber.
Mitochondrial biogenesis happens when your body senses a need for more energy and kicks off a chain reaction inside muscle cells. First, energy “sensors” (ex: AMPK, AMP-activated protein kinase aka AMPK is a protein that monitors the energy levels in cells and helps maintain homeostasis) activate a master switch called PGC-1α, which travels to the nucleus and helps turn on genes for building mitochondrial parts (like enzymes and membrane proteins). New mitochondrial DNA is then copied, and all these freshly made proteins are transported into growing mitochondria, which split and merge to expand the network, ending up with a larger, more powerful population of energy-producing organelles.
A higher mitochondrial count translates to an increased capacity to produce adenosine triphosphate (ATP) via oxidative phosphorylation, meaning that the body can generate energy at a faster rate using oxygen as the primary driver. Concomitantly, the enzymatic machinery within these mitochondria becomes more efficient, as indicated by increases in the activity of oxidative enzymes such as citrate synthase and cytochrome c oxidase (Holloszy & Coyle, 1984). We’ve talked about this in Training for the New Alpinism, Training for the Uphill Athlete, in this article, and as it pertains to nutrition in this article.
Bottom line? With a higher density of mitochondria and more active enzymes, athletes gain a notable advantage in sustaining moderate to high workloads without fatiguing as quickly.
With a higher density of mitochondria and more active enzymes, athletes gain a notable advantage in sustaining moderate to high workloads without fatiguing as quickly.
LACTATE METABOLISM
Against this backdrop of mitochondrial enhancement, lactate metabolism presents another compelling target for improving endurance. Historically, lactate was viewed as a simple byproduct of anaerobic metabolism responsible for acidosis, but researchers such as Brooks (1985) and more recently, Gladden (2004), have shown that lactate plays a far more dynamic role. When muscles are active at higher intensities, they produce lactate, which can then be released into the bloodstream or shuttled to adjacent muscle fibers. In well-trained individuals, the capacity to take up and oxidize this lactate (use it as a fuel) increases significantly due to an upregulation of transport proteins called monocarboxylate transporters (MCTs). These proteins permit lactate to cross cell membranes, where it is then converted back to pyruvate by lactate dehydrogenase (LDH) enzymes before entering the Krebs cycle (also known as the citric acid cycle) for further energy extraction. This process—often referred to as the “lactate shuttle”—enables athletes to use lactate as a viable fuel source rather than what was once thought to be a metabolic dead end.
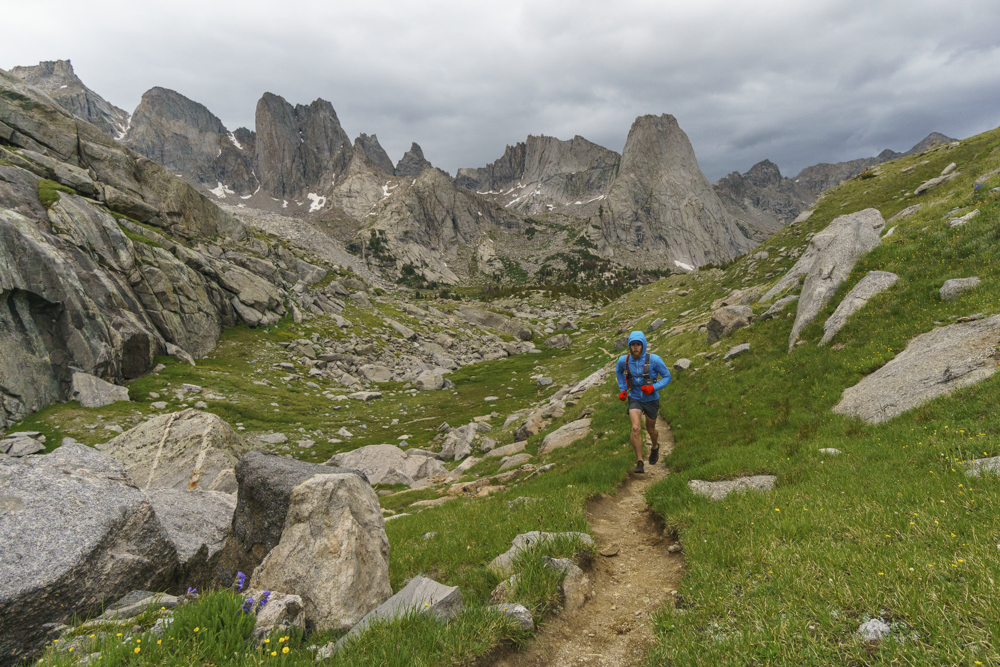
The ability to oxidize lactate effectively is intimately linked to specific training-induced changes. First, an increase in mitochondrial density gives muscle fibers a larger metabolic engine, effectively expanding their capacity to handle substrates like lactate (Holloszy & Coyle, 1984). Second, the heightened expression of MCTs—especially MCT1—is crucial for facilitating lactate uptake into mitochondria (Bonen, 2001). Third, the balance of LDH isoforms shifts in a way that favors the conversion of lactate into pyruvate over pyruvate into lactate, reinforcing the role of lactate as a fuel rather than a byproduct (Gladden, 2004). These coordinated adaptations help well-trained endurance athletes not only clear lactate more quickly but also use it to power continued muscle contraction, delaying the onset of muscular fatigue.
In practice, these physiological insights translate to training strategies that prioritize long, steady bouts of aerobic work—commonly known as Zone 2 training—along with appropriate recovery periods. Prolonged, lower-intensity sessions stimulate mitochondrial biogenesis, upregulate oxidative enzymes, and maintain a low enough level of muscular stress to allow for effective lactate shuttling. Such sessions lay the groundwork for broader metabolic flexibility, as the body becomes adept at both burning fat at lower intensities and oxidizing lactate when the intensity rises. Athletes who neglect these foundational sessions often find themselves lacking the aerobic base necessary to fully capitalize on more intense workouts, ultimately leaving performance gains on the table.
Equally important is adequate recovery, during which the cellular changes from endurance training truly manifest. When training load exceeds the body’s capacity to adapt, the benefits of mitochondrial proliferation and improved lactate transport can be partially lost—or at least blunted—by excessive fatigue. (We’ve written about overtraining here, here, and here.) By balancing hard efforts with rest days or recovery efforts, athletes allow the physiological remodeling that underpins these adaptations to take hold, culminating in a more robust aerobic engine. Proper rest, alongside disciplined nutrition and sleep, helps reinforce the shift toward more oxidative forms of LDH, supports the maintenance of high MCT expression, and stabilizes the gains made from consistent Zone 2 training.
Conclusion
Ultimately, recognizing that lactate serves as both a marker and a fuel for performance supports our scientific understanding of how athletes should approach their training. Rather than thinking that lactate accumulation is nothing more than a sign of imminent fatigue, well-conditioned endurance athletes come to see it as a resource that—when properly managed—can help extend work output and stave off exhaustion. The synergy between mitochondrial biogenesis and lactate utilization underlines why steady aerobic efforts and deliberate recovery must stand at the core of a well-rounded program. By investing in these fundamental processes and appreciating how lactate can be leveraged as an energy source, athletes cultivate the physiological resilience required to excel in long-duration, high-aerobic-demand endeavors in the mountains and on the trails.
REFERENCES
- Bonen, A. (2001). The expression of lactate transporters (MCT1 and MCT4) in heart and muscle. European Journal of Applied Physiology, 86(1), 6–11.
- Brooks, G. A. (1985). The lactate shuttle during exercise and recovery. Medicine and Science in Sports and Exercise, 17(1), 22–31.
- Gladden, L. B. (2004). Lactate metabolism: a new paradigm for the third millennium. The Journal of Physiology, 558(1), 5–30.
- Holloszy, J. O., & Coyle, E. F. (1984). Adaptations of skeletal muscle to endurance exercise and their metabolic consequences. Journal of Applied Physiology, 56(4), 831–838.